Introduction
Materials and Methods
Plant collection and pigment extraction
Analyses of pigment compound
Antioxidant assay
Assay for anticancer activity
Results
Plant morphology in different environmental conditions
Extraction of pigment compound
UV-Vis spectra
Stability studies on pH, temperature, and light
Gel electrophoretic migration pattern
LC-MS pattern
Antioxidant activity
Anticancer activity
Discussion
Introduction
The halophytic plants (halophytes) are characterized by the high adaptability against high-level ionic concentration and are classified into facultative and obligatory halophytes (Flowers et al., 1986). Facultative halophytes (e.g. many species of Poaceae, Cyperaceae, Juncaceae; Glaux maritima, Plantago maritima, Aster tripolium) live in a slightly saline soil, but their growth is easily inhibited by the elevated salt levels. Obligatory halophytes (euhalophytes, e.g. Salicornia, Salsola, Suaeda, Halocnemum) are confined to saline habitats, and their growth is promoted by the uptake of moderate amounts of salt; only when the salt reaches to a very high level is growth impaired with the signs of stress, such as the production of pigments or the breakdown of chlorophyll (Larcher, 1995). However, it was reported that these plants can spontaneously or artificially grow in low-saline soils (Barbour and Michael, 1970; Barbour et al., 1987). Plants growing in saline habitats cannot evade the effects of salt and should therefore develop at least some degree of salt tolerance either by keeping excessive amounts of salt from protoplasm or by eliminating the toxic and osmotic effects caused by the high ionic concentrations (Flowers and Colmer, 2008). Halophytes found in the species of Salicornia, Suaeda, and Atriplex compensate for the high osmotic potentials by accumulating salt in the cell sap. When these plants absorb salt, they intake water and become chubby, thereby decreasing salt concentration. This condition induces development of succulence that is generally shown in halophytic plants. Some other halophytic plants have a different mechanism of salt tolerance. For example, the cells of root endodermis make up wax layer and do not pass salt (NaCl) (Glenn et al., 1998). Some dicotyledonous halophytes, such as species of Salicornia and Suaeda, have succulent leaves composed of enlarged cells in which the vacuoles occupy most of the cell volume. A few compatible solutes are known to be glycine betaine, proline, polyols and cyclitols, some of which are not only benign but also protective to enzymes (Pollard and Wyn Jones, 1979).
Many halophytic Suaeda species have been reported as utilizable plants. The S. salsa was utilized as food, medicine, forage and bioenergy, and this plant plays an important role in the restoration of salinized or contaminated saline land (Song and Wang, 2015). The S. australis extract showed marked antibacterial activities (Kim et al., 2016). The S. maritima extract showed the anti-Toxoplasma gondii effect (Hong et al., 2014) and had excellent antioxidant effects (Kim et al., 2016). The S. japonica has been used as a functional food and a medicinal plant due to its beneficial effects including α- glucosidase inhibition and antioxidant and anti-diabetic activities (Kang et al., 2017). The S. japonica belongs to the family Chenopodiaceae. As an annual herbage it is a representative obligatory halophyte that can grow in high salt concentration. These plants live in the mudflat at intertidal zone of western and southern coastal areas of Korea and exhibit a characteristic purple color in leaf and stem. They remain submerged in seawater during the most of their life. However, it was found that a few of the same species also grow in terrestrial soil nearby, but not in mudflat, without being soaked in seawater. They appear to be produced by the seeds transferred from mudflat during the process of embankment construction. These plants no longer contact with seawater and grow in freshwater soils, although their habitats are limited to a particular area. These plants may have a natural ability to survive in both saline and non-saline environments (Barbour and Michael, 1970).
Studies on Suaeda species may give a new insight into a mechanism of the salt tolerance as well as the saline adaptation (Ayarpadikannan, 2012; Alhdad et al., 2013; Flowers and Colmer, 2015; Xu et al., 2017). Although some mechanisms such as the accumulation of salts in leaf vacuoles have been shown in halophytic plants, little is known about importance of pigment compound that is associated with the halotolerance in Suaeda species. As one of the steps toward understanding how the plant is well adapted to strongly saline habitats, the morphological differences between seawater-living and freshwater- living S. japonica plants were compared. The role of peculiar purple pigment compound as a probable osmolyte in the saline environment was also discussed. Besides, the pigment compound that is highly accumulated in leaves was extracted and examined its basic characteristics such as the water solubility, pH- and temperature-dependent color changes, sensitivity to light, UV-Vis spectra, gel electrophoretic migration pattern, LC-MS pattern, antioxidant activity, and anticancer activity against several tumor cell lines in this study.
Materials and Methods
Plant collection and pigment extraction
The plants (Suaeda japonica Makino) were collected in mudflat (seawater-living plants) and terrestrial soil nearby (freshwater- living plants) at Ha-Ri, Samsan-Myeon, Gangwha-Gun, Incheon, Korea. They were washed in distilled deionized water, blotted onto paper towels, and immediately used for pigment extraction or stored at -70℃ until use. For adaptation to terrestrial environment, some seawater-living plants were transferred to the potting soil and watered with freshwater.
The pigment compound was extracted in 5 ㎖ water per gram fresh leaves as described essentially in Chung and Byun (2009). Briefly, after grinding in mortar with pestle, they were centrifuged at 15,000 rpm for 10 min and the supernatant was transferred to the fresh vials. The water-extracted pigment compound was further treated with ethyl acetate, ethyl ether, and chloroform successively to remove other organic substances. The extracted pigment solution could be further concentrated to the solid state by butanol extraction and air dried, and finally used as the sample of all experiments in this research.
Analyses of pigment compound
To observe the absorption spectrum of the extracted purple pigment, wavelength (200 to 700 ㎚) was scanned by UV-Vis spectrophotometer. The effects of pH, temperature, and light on pigment stability were performed with pigment samples that were dissolved in H2O (0.5 ㎎/㎖). For the stability studies on pH, the pigment samples were kept at different pH values (ranging from pH 1, pH 2, pH 3, pH 6, pH 7, pH 8, pH 9, pH 10, and pH 11) at 25℃ for 12 h and observed. For the stability studies on temperature, the pigment samples (pH 7) were kept at different temperature conditions (ranging from 25℃, 45℃, 65℃, and 85℃) for 12 h and observed. The color change of pigment sample (pH 7) was also observed under the exposure to daylight during two weeks.
To clarify whether the purple pigment belongs to anthocyanin or betacyanin that are two major representative water-soluble pigment compounds in plants, the extracted pigment sample was migrated on a horizontal slab gel electrophoretic apparatus (Chung and Byun, 2009). Electrophoresis of purple pigment was carried out in a citrate buffer (10 mM citric acid anhydrous, 3 mM sodium citrate dihydrate, pH 3.0) on 3.0% agarose gel. Already well known two kinds of water- soluble plant pigments (anthocyanins and betacyanins) were used as standard references. Standard anthocyanin pigments were extracted from leaf of maple (Acer palmatum, Fossen and Andersen, 1999), fruit of blueberry (Vaccinium corymbosum, Lohachoompol et al., 2008), flowers of morning glory (Pharbitis nil, Saito et al., 1996), balloon flower (Platycodon grandiflorum, Goto et al., 1983), and leaf of begonia (Begonia semperflorens, Chirol and Jay, 1995). Standard betacyanin pigments were extracted from root of red beet (Beta vulgaris, Strack et al., 2003), flowers of cockscomb (Celosia cristata, Schliemann et al., 2001), flowers of four o'clock (Mirabilis jalapa, Gandía-Herrero et al., 2005), and fruit of pokeweed (Phytolacca americana, Schliemann et al., 1996).
LC-MS (liquid chromatography-mass spectrometry) analysis of the extracted pigment compound was performed at the Korea Basic Science Institute (Daejeon, Korea) using a Instrument [LCQ DECA XPplus (Thermo Finnigan, UK ; Ionization, Micro flow-ESI (+) ; Column, C18 ; Mobile phase, 100% water (0.5% acetic acid / 0.02% formic acid) / 80% acetonitrile (0.5% acetic acid / 0.02% formic acid) ; Sample solvent, 50% methanol].
Antioxidant assay
The free radical scavenging activity of the pigment compound was assayed using a stable DPPH (1,1-diphenyl- 2-picryl-hydrazyl) radical, following standard method (Blois, 1958; Kim et al., 1981). The DPPH solution (0.08 ㎎/㎖) was prepared in 50% ethanol. The pigment sample solutions dissolved in 50% ethanol were prepared in different concentrations (0.3 to 9 ㎎/㎖). The reaction mixture contained 1.5 ㎖ of DPPH solution and 0.3 ㎖ of sample solution. The absorbance was read at 528 ㎚. The readings were measured at different time intervals for a total duration of 30 min. The DPPH, which forms a deep violet color in solution, reacts with antioxidants and color loss at 528 ㎚ correlates to antioxidant content. BHA (butylated hydroxyanisole) was used as control. Each test was carried out twice and the absorption value was averaged.
Assay for anticancer activity
The anticancer activity and the resulting data analysis were performed essentially as described by Choi et al. (2006) following the MTT [3-(4,5-dimethyl-thiazol-2-yl) -2,5-diphenyl- tetrazolium bromide] assay (Rubinstein et al., 1990). The human tumor cell lines used were A549 (Korean Cell Line Bank No. 10185, lung carcinoma), HEC-1B (ATCC No. HTB- 113, uterus adenocarcinoma), SW-156 (ATCC No. CRL-2175, kidney hypernephroma), and T98G (Korean Cell Line Bank No. 21690, brain glioblastoma multiformae). Cells undergoing exponential growth were suspended in a fresh PBS (phosphate buffered saline) medium at a concentration of 2×105 cells/㎖, dispensed in a 96-well flat plate in a volume of 0.1 ㎖ per well, and stabilized by incubation at 37℃ in a 5% CO2 for 24 h. Then, 100 ㎕ each sample was added to the wells. After incubation at 37℃ for 44 h, 50 ㎕ of MTT (0.5 ㎎/㎖ PBS) was added to each well, and the resulting 250 ㎕ mixture was further incubated for 4 h at 37℃. The formazan crystals formed in each well were dissolved in 100 ㎕ of dimethylsulfoxide solution by mixing with plate shaker. The optical density of the colored reaction sample was measured by reading at 570 ㎚ on a multi-well scanning spectrophotometer.
Results
Plant morphology in different environmental conditions
The S. japonica is an annual herbaceous dicotyledonous plant and the height of a mature plant is approximately 30 ㎝. The seawater-living plants showed collectively purple color in leaves that are distinctively succulent and extended (Fig. 1 A and B), whereas the freshwater-living plants showed collectively green color in leaves that are less succulent and somewhat more extended (Fig. 1 C and D). When seawater-living plants were transferred to potting soil and watered with freshwater for adaptation to the terrestrial environment, the purple color was gradually changed to green color in the leaves (Fig. 1E). They were morphologically very similar to the freshwater-living plants and grew slightly faster than seawater-living plants. The seawater-living plants showed a peculiar purple color during their whole life. In contrast, both freshwater-living plants and freshwater-adapted seawater-living plants lost the purple color almost completely and displayed a green color in leaves like the glycophytes (Fig. 1 C, D, and E).
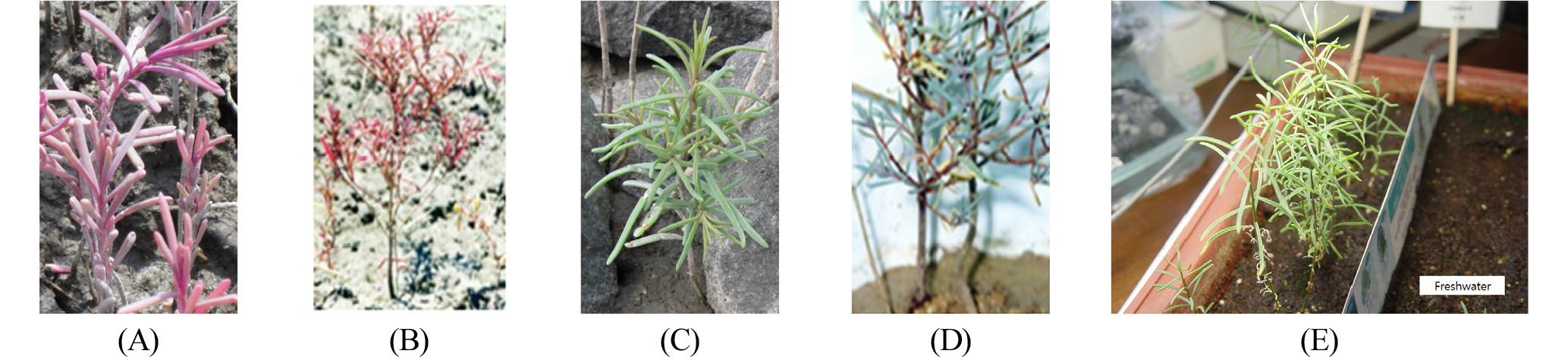
Fig. 1.
Morphologies of S. japonica in saline and non-saline environments. A and B, Young and fully grown seawater-living plants at the mudflat. C and D, Young and fully grown freshwater-living plants at the terrestrial soil nearby. E, Freshwater-adapted seawater-living plants after transfer to the potting soil.
Extraction of pigment compound
Various solvents were attempted to extract the purple pigment compound from leaf tissues. The purple pigment was found to be best extracted in water. No purple pigment was virtually extracted from freshwater-living plants. Fig. 2 shows the water-extracted pigments after blending with various solvents. The purple pigment was completely distinguished from the photosynthetic green pigment, chlorophyll, as can be seen in ethyl acetate or ethyl ether solvents in Fig. 2A. The purple pigment compound was insoluble in ethyl acetate, ethyl ether, chloroform, benzene, and hexane (Fig. 2A). The extracted pigment solution could be further concentrated to the solid state (Fig. 2B).
UV-Vis spectra
To observe the absorption spectrum of the extracted purple pigment, it was measured in both UV and visible light ranges (200 ㎚ to 700 ㎚). As shown in Fig. 3A, the absorbance maxima occurred in the UV range and in the visible range at near 540 ㎚. However, the absorption maximum at 540 ㎚ of purple pigment was disappeared under alkaline condition (Fig. 3B).
Stability studies on pH, temperature, and light
The variation of color and stability of the compound was observed when pH or temperature was changed (Tables 1 and 2). The pigment color was purple in acidic conditions, but became yellowish in alkaline conditions. As the pH of acidic purple pigment solution was raised, the color was slightly changed to reddish purple up to pH 7, but became yellowish at above pH 8. This result indicates that the pigment is relatively stable up to pH 7. The pigment compound was stable (reddish purple) at room temperature (25℃) under the pH 7 condition, but was slightly unstable (pale purple) at 45℃ to 85℃. When the purple color of water-extracted pigment compound was exposed to daylight, it was gradually faded and became yellowish after 14 days (Fig. 4).
Table 1. pH-dependent color change and stability of pigment at 25
pH | Color | Stabilityz |
1 | Deep Purple | +++y |
2~3 | Purple | ++ |
6~7 | Reddish Purple | + |
8~9 | Colorless/Pale Yellow | - |
9~10 | Pale Yellow | -- |
10~11 | Yellow (ppt)x | --- |
y+ and - denote stability and unstability, respectively.
xppt = precipitated.
Table 2. Temperature-dependent color change and stability of pigment at pH 7
Temp | Color | Stabilityz |
25℃ 45℃ 65℃ 85℃ | Reddish Purple Pale Purple Pale Purple Pale Purple | +y - - - |
y+ and - denote stability and unstability, respectively.
Gel electrophoretic migration pattern
When the standard anthocyanin references (maple, blueberry, morning glory, balloon flower, and begonia) and the standard betacyanin references (red beet, cockscomb, four o'clock, and pokeweed) were separated together on an agarose gel at the same time, they were migrated to opposite directions; the anthocyanins to cathode while the betacyanins to anode (Fig. 5). The purple pigment of S. japonica (lane 10) was moved to anode along with the standard betacyanin references (lanes 2, 4, 6, and 8) as can be seen in Fig. 5, indicating that this pigment belongs to betacyanin.
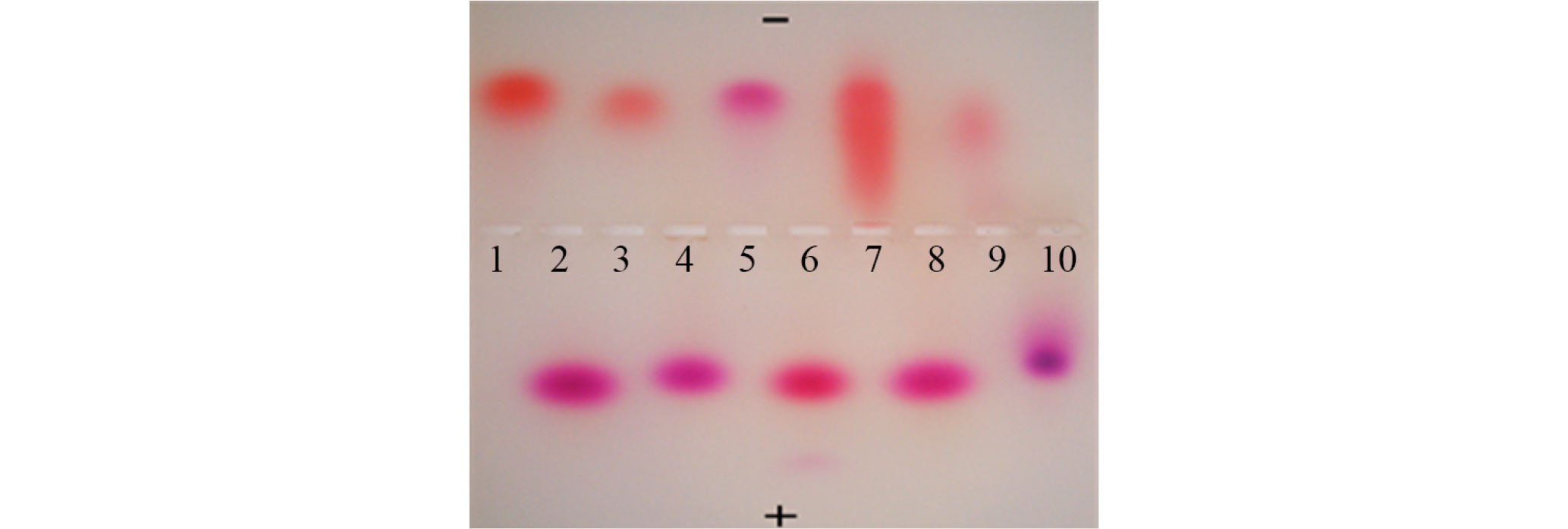
Fig. 5.
Gel electrophoretic migration patterns of anthocyanins and betacyanins in citrate buffer.
1. Begonia (Begonia semperflorens) anthocyanin
2. Pokeweed (Phytolacca americana) betacyanin
3. Maple (Acer palmatum) anthocyanin
4. Cockscomb (Celosia cristata) betacyanin
5. Balloon flower (Platycodon grandiflorum) anthocyanin
6. Red beet (Beta vulgaris) betacyanin
7. Morning glory (Pharbitis nil) anthocyanin
8. Four o'clock (Mirabilis jalapa) betacyanin
9. Blueberry (Vaccinium corymbosum) anthocyanin
10. Suaeda japonica this study
LC-MS pattern
LC-MS analysis of the extracted pigment compound was performed. As shown in Fig. 6, total ion chromatogram showed two major peaks at 21.32 min and 28.41 min of the retention time (A). The extracted ion chromatogram of each peak showed the protonated molecular ions at m/z 651.1 (B) and 827.1 (C), respectively.
Antioxidant activity
Antioxidant activity of the extracted pigment compound was determined by DPPH assay (Table 3). The pigment compound was found to have an antioxidant activity that is linearly increased in proportion to the reaction time for up to 30 min, and the activity was comparable to that of control BHA at 9.0 ㎎/㎖.
Table 3. Antioxidant activity of the pigment compound (unit: OD at 528 ㎚)
Anticancer activity
The anticancer activity against several tumor cell lines was also examined following the MTT assay (Table 4). The significant growth inhibitory effect was observed on two tumor cell lines SW-156 (human kidney hypernephroma) and HEC- 1B (human endometrial adenocarcinoma).
Table 4. Growth inhibitory effects on tumor cell lines
Conc. (㎎/㎖) | Cell Linesz | |||
A549 | HEC-1B | SW-156 | T98G | |
10.0 5.0 2.5 1.0 | 48.0y 23.7 26.1 15.7 | 58.4 29.5 22.9 - | 70.5 66.6 35.2 - | 18.5 -x 2.4 - |
yNumbers in each column indicate the percentage of cell growth inhibition.
x- denotes no data.
Discussion
Influenced by external conditions of habitat, halophytic plants generally have a characteristic morphology that distinguishes from glycophytic plants. The S. japonica plant used in this study has a characteristic purple colored succulent leaves during the life cycle in saline environment. Among the treated solvents to extract the purple pigment, the water was the best. This result was consistent with that of the betacyanin extraction from Opuntia fruit (Castellar et al., 2003). The extracted pigment compound color was gradually faded out and became yellowish after exposure to 14-daylight (Fig. 4), indicating that the pigment compound is unstable and destroyed by light once isolated from the plant. It has been reported that light causes betacyanin degradation (Woo et al., 2011). The pigment color was purple in acidic conditions, but became yellowish in alkaline conditions. According to the report of Jackman and Smith (1996), the betacyanins keep their appearance over the broad pH range from 3 to 7, but degrade at above pH 9. In the present study, the purple pigment color of S. japonica was changed to yellow at above pH 8 (Table 1). The pigment color was relatively stable at the pH range from 1 (deep purple) to 7 (reddish purple), but unstable at alkaline conditions (yellowish). When the pigment samples at pH 7 (reddish purple) were raised to the various levels of temperature with 20℃ intervals, the colors were slightly changed to pale purple at 45 to 85℃, indicating that the pigment is relatively stable at high temperatures (Table 2). Betacyanins are known to be light-sensitive, pH-dependent of color change, and easily hydrolyzed at alkaline pH (Jackman and Smith, 1996; Stintzing and Carle, 2004). Therefore, the color change from purple to yellow (Table 1) indicates that the pigment of S. japonica is betacyanin, because betacyanins are degraded under alkaline conditions while the another water-soluble anthocyanins are known to blue under alkaline conditions (Cabrita et al., 2000; Jackman and Smith, 1996). Since the extracted purple pigment was most stable at acidic pH, this pigment might be stored in acidic vacuoles (pH 2.7 to pH 7.7) where pigments are known to be located (Nelson and Harvey, 1999).
The Fig. 3 displays the typical UV-Vis spectra of betacyanin when compared with those of other reports (Cai et al., 1998; Woo et al., 2011). The absorption peak ranging from 530 to 545 ㎚ is a spectral characteristic of the betacyanins (Castellar et al., 2003; Allegra et al., 2005; Tsai et al., 2010). The absorption maximum at 540 ㎚ of purple pigment was disappeared as shown in figure 3B, suggesting that betacyanins are degraded under alkaline conditions. This result is consistent with that of the color changes under alkaline pH conditions (Table 1). The betacyanins are difficult to separate from protein. According to the report of Cai et al. (1998), the betacyanins have a special relationship with protein. When the UV-Vis spectral determination of diverse Amaranthus betacyanins was conducted, they observed that even the purified samples had also abnormally strong absorbance at the range of UV light. The purified betacyanins using the procedures by (NH4)2SO4 salting-out, HCl-treatment, centrifugal separation, and isolation by Sephadex G-25 and G-50 columns to remove proteins still contained protein. They concluded that the betacycnins could not readily be completely isolated from the protein. Likewise, the UV spectral peaks of purple pigment as shown in Fig. 3 seem to be due to the typical UV absorbance spectra of unseparated protein or other contaminated components. Betacyanins in plants usually are in the form of glycosylated betanidin derivatives. They are also well known to display two absorption maxima, one in the UV-range due to the cyclo- DOPA (cyclo-3,4-dihydroxyphenylalanine) structure and a second one in the visible range. Besides, acylated betacyanins display an additional absorption in the UV region of about 300 to 330 ㎚, where the absorption of nonacylated betacyanins is very weak (Cai et al., 2001; Stintzing and Carle, 2004). In this study, the purple pigment compound of S. japonica displayed an extra absorption maximum in the UV range at near 330 ㎚, suggesting that the pigment compound is probably acylated. The absorption maximum values of the betacyanins can be slightly changed due to the structural differences. Generally, the red and violet colors in betalain-bearing plants are produced by different substitution patterns of betacyanins. For example, betacyanins are formed of a betalamic acid unit linked to a molecule of cyclo-DOPA. The latter highly aromatic structure is responsible for the deep violet color for the pigment and this aromatic structure also induces a strong bathochromic shift of 60-70 ㎚ (absorption max. 534-554 ㎚) (Wyler and Dreiding, 1961; Strack et al., 2003). The absorption maximum of purple pigment in the present study was 540 ㎚ in the visible range and the characteristic purple color may be also due to the betacyanin-specific cyclo-DOPA structure.
To clarify furthermore if this purple pigment of S. japonica belongs to anthocyanin or betacyanin, an another novel method by agarose gel electrophoresis (Chung and Byun, 2009) was additionally applied. At acidic pH 1 to 3, anthocyanins exist chiefly in the form of the flavylium cation (Goto et al., 1976; Tanaka et al., 2008) and occur in the most intense reddish colors. As the pH increases, deprotonation occurs so that at neutral pH, anionic forms of the quinonoidal base predominate (Cabrita et al., 2000; Jang et al., 2005). On the other hand, the charge of betacyanin becomes positive at pH less than 1.5, negative at pH between 2.5 and 9.5, and neutral (zero charge), an isoelectric point, at pH between 1.5 and 2.5 (Yang et al., 2003). Therefore, using the citrate buffer (pH 3.0) in this study, most of the anthocyanin molecules will be in the positively charged flavylium forms, while the betacyanin molecules will be in the negatively charged forms (Chung and Byun, 2009). With oppositely charged ions they will migrate to the opposite directions. Indeed, the extracted standard pigment samples (anthocyanins and betacyanins) from various plant species in the present study were moved to the opposite directions on an agarose gel in citrate buffer (pH 3.0); anthocyanins to cathode and betacyanins to anode (Fig. 5). The purple pigment of S. japonica was moved to anode along with other standard betalcyanins extracted from red beet, four o'clock, pokeweed, and cockscomb. This result also strongly suggests that the purple pigment of S. japonica is betacyanin, but not anthocyanin.
In addition, betacyanins have been exclusively found in the family Chenopodiaceae. Moreover, anthocyanins and betacyanins have never been found together in the same plant and intermolecular copigmentation phenomena with betacyanins have not been reported so far (Stafford, 1994; Stintzing and Carle, 2004; Tanaka et al., 2008). Their structural difference is based on divergent biochemical pathways on the arogenate level, the precursor of tyrosine (betacyanins) and phenylalanine (anthocyanins), respectively (Steglich and Strack, 1990; Tanaka et al., 2008).
Betacyanins have been extracted from various plant sources. Among the betacyanins present in plants include betanin, isobetanin, probetanin, and neobetanin. Other important betacyanins are amaranthine and isoamaranthine, isolated from species of Amaranthus. The betacyanin isolated from Christmas cactus flowers (Schlumbergera x buckleyi) showed in LC-MS a protonated molecular ions ([M + H]+) at m/z 637, 769, and 945 (Strack et al., 2003). In LC-MS, the protonated molecular ions of betacyanins were found at m/z 727 and 829 in fruit extracts of pokeberry (Phytolacca americana), at m/z 695 in pigment of vine cactus (Hylocereus polyrhizus), and at 727, 871, 872, and 903 in extracts of cockscomb (Celosia argentea) (Strack et al., 2003). The betacyanin of Djulis (Chenopodium formosanum) exhibited the maximum absorbance at 530 ㎚ and showed four peaks including betanin (m/z 551.0), isobetanin (m/z 551.0), amaranthine (m/z 727.0), and isoamaranthine (m/z 727.0) (Tsai et al., 2010). The major mass difference of betacyanins is due to the presence or absence of additional sugar moiety (Strack et al., 2003). The LC-MS analysis (Fig. 6) of the S. japonica pigment compound showed two major peaks with the protonated molecular ions at m/z 651.1 and 827.1, indicating that the pigment compound may be composed of at least two major betacyanin components. Further analysis is required to find the unknown chemical structures of these two compounds. Antioxidant activity of the pigment compound was also identified. The activity was comparable to that of control BHA at 9.0 ㎎/㎖ (Table 3). If the pigment were more highly purified by applying another additional biochemical procedures, the activity would be increased much more at the lower concentrations. Betacyanins are present in a large number of plants, vegetables, flowers, and fruits and have been reported for their antioxidant activities (Betancourt et al., 2017). The natural betacyanins are increasing in importance for food industry applications and are replacing a number of synthetic colorants that have been questioned and dropped from the market due to safety issues. Betacyanins extracted from different vegetable sources are bioavailable and have been reported for their antioxidant protective activities that include inhibition of lipid peroxidation and heme decomposition, and inhibition of oxidative metabolism in neutrophils from obese individuals (Caldas-Cueva et al., 2015). As shown in Table 4, the pigment compound displayed inhibitory activity toward human tumor cell lines. Especially, the significant growth inhibitory effect was observed on SW-156 (human kidney carcinoma) and HEC-1B (human endometrial adenocarcinoma). Therefore, it seems that the S. japonica pigment compound could be also utilized for human health.
Taken together, the purple pigment compound of S. japonica exhibits typical characteristics of betacyanin that were represented by water solubility, pH- and temperature-dependent color changes, sensitivity to light, UV-Vis spectra, and gel electrophoretic migration pattern. The purple pigment might be also involved in the halotolerance, since it is specifically accumulated in high abundance in seawater-living plants (Fig. 1 A and B), but disappeared in freshwater-living plants (Fig.1 C and D) as well as in freshwater-adapted seawater-living plants (Fig. 1E). In another representative halophytic plant Mesembryantheum crystallinum, the production of betacyanin was usually associated with the salt (NaCl)-stressed state and the betacyanin was already regarded as an osmolyte (Adams et al., 1998). In Salicornia europaea L., the betacyanin synthesis was induced as a result of salt stress (Bothe, 1976), suggesting that betacyanins function as osmolytes to uphold physiological processes in the halophytic plant (Stintzing and Carle, 2004). Similarly, the purple pigment compound (betacyanin) of S. japonica may also function as an osmolyte to uphold halotolerant physiological processes in saline environment. In conclusion, this study reveals the presence of the purple betacyanin compound that may enable this halophytic plant to tolerate saline environment in which it can function as a probable osmolyte without any significant harmful effects on plant growth and development. The purple pigment shows also that it can function as antioxidant and anticancer agents.